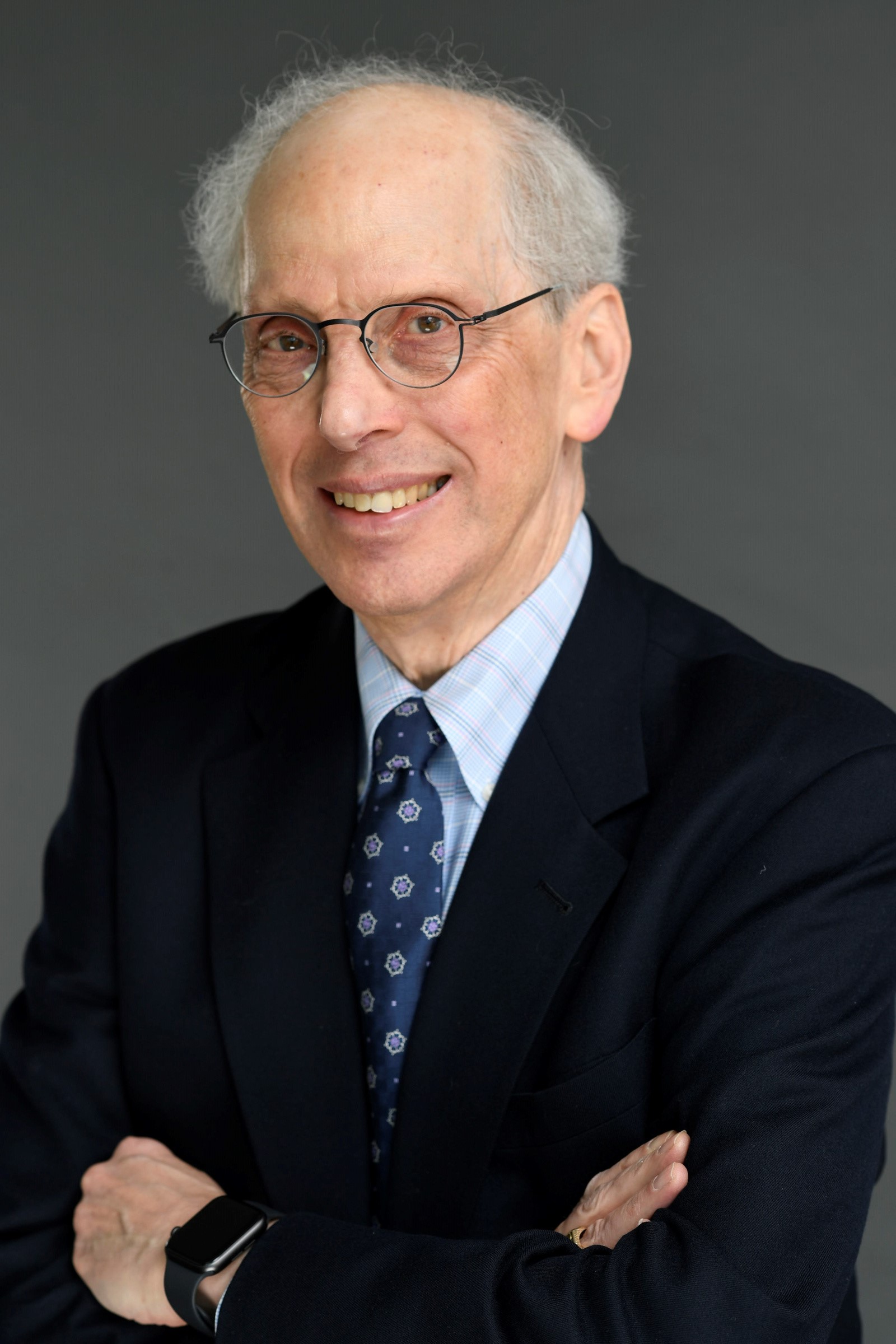
CQE PI Feature – Jeffrey H. Shapiro
From ham radio to optical and quantum communication via Fourier optics
Featured in QSEC October newsletter 2024
In high school, my two best buddies and I became interested in amateur (“ham”) radio. We got our licenses and did some electronics tinkering, but the main attraction was contacting other hams from all over the country and the world. Nevertheless, ham radio propelled us to study electrical engineering when we went to college, which for me was at MIT starting in 1963. Sophomore EE subjects in circuits convinced me that circuits would not be my thing. Moreover, the sophomore Physics subject on optics made that field even less appealing than circuits. The junior year EE subject in signals and systems, however, really turned me on. The power and beauty of linear systems analysis resonated with me, e.g., in understanding the principles of a single-sideband phasing transmitter. As a senior I took the Principles of Communication class, and became enthralled by the noise analysis used in quantifying communication performance. Thus, after entering MIT graduate school on an NSF fellowship, I sought out Prof. Robert Kennedy — my instructor in Principles of Communication — to find a Master’s thesis. He was building up a group to apply communication theory to optics, motivated by the possibilities afforded by lasers. I had some trepidation about joining the group, but then I went to some introductory lectures for incoming students. There I saw diffraction expressed as a 2D convolution and the imaging performance of ideal lens characterized by a 2D spatial-frequency response. After that, I was all-in on optical communication. For me, the rest was history — MIT Ph.D. in 1970, 3 ½ years as an Assistant Professor at Case Western Reserve University, and on the MIT faculty since 1973 — doing research on a wide swath of topics at the juncture of physics and communication, including imaging and precision measurements in addition to information transmission.
The research in Kennedy’s group initially focused on two ways in which communication at optical frequencies differs from communication at radio frequencies, viz., the importance of spatial effects in atmospheric optical communication, and the role of quantum mechanics in determining ultimate limits on optical communication. My doctoral thesis, on optical communication through atmospheric turbulence, included the first proof that the turbulent channel was reciprocal, the principle that underlies adaptive-optics correction for satellite uplinks. The doctoral thesis of Horace Yuen, another Kennedy graduate student at that time, included the solution to the M-ary hypothesis-testing problem for non-commuting density operators, which required what are now called positive operator-valued measurements as opposed to just observables. Horace and I returned to MIT in 1973, and we began to collaborate on using two-photon coherent states — now called squeezed states — for optical communication. Our collaboration led to a series of papers in the IEEE Transactions on Information Theory. One established the quantum theory of Fresnel diffraction, enabling arbitrary quantum states to be propagated for communication and imaging analyses. Another proved the Yuen-Shapiro representation theorem for quantum photodetection, providing continuous-time, continuous-space, operator-valued representations for direct, homodyne, and heterodyne detection. We also published a paper in Optics Letters that proposed four-wave mixing as a means to generate squeezed states. That technique was used by Dr. Richart Slusher to make the first successful demonstration of quadrature-noise squeezing in 1985.
By 1980 or so it was clear that I needed an experimentalist in my group with whom to collaborate. At first it was Dr. Prem Kumar, who served on the RLE Research Staff until 1986 and is now a senior faculty member at Northwestern University. Since then it has been Dr. Franco Wong, who is now a Senior Research Scientist in RLE. Over the last 40+ years theory has sometimes led experiment and experiment has sometimes led theory in RLE’s Optical and Quantum Communication Group. Early squeezing experiments gave way to generating polarization-entangled photon pairs and two-mode squeezed-vacuum light beams, and then applying them to sensing, imaging, and communication. Much attention was paid to the boundary between classical and quantum optics, e.g., in distinguishing quantum ghost imaging from pseudothermal ghost imaging, which led us to develop the theory of phase-sensitive coherence. Two-mode squeezed-vacuum radiation, used in the quantum illumination protocol, was shown to outperform its best classical competitor in detecting a weakly-reflecting target embedded in entanglement-breaking background radiation. The quantum illumination idea was later modified to show how to communicate with complete immunity to passive eavesdropping. We showed that similar quantum-protected behavior could be obtained were low-brightness amplified spontaneous emission noise used for transmission, while a high-brightness version of that classical-state light was retained for measurement purposes. This realization led to the theory and demonstrations of floodlight quantum key distribution and quantum low probability of intercept.
The vast majority of my quantum work has been devoted to communication, sensing, and imaging, but I have made occasional forays into quantum computation. My 2006 Physical Review A article, showing that causality-induced phase noise might preclude Kerr-nonlinearity single-photon two-qubit gates, continues to draw references. In 2018, with our doctoral student Murphy Yuezhen Niu as lead author, Prof. Isaac Chuang and I published two papers showing that linear optics plus (2) interactions with Fock-state pumps could realize a universal gate set in qudit bases, and proposing hardware-efficient bosonic quantum error-correcting codes for quantum computation based on (2) interactions. My latest work, still ongoing, is performance analysis for zero added-loss multiplexing, a quasi-deterministic heralded source of polarization-entangled biphotons for dramatically increasing the rate of entanglement distribution.
When you get to my career stage — the verge of retirement — looking back rather than ahead is always a temptation, and I have certainly fallen prey to it here. Thus let me close by saying the future of quantum communication, sensing, and imaging is very bright at this time. Exciting ideas abound in all these domains. Entanglement distribution enhanced by the emergence of quantum repeaters will ultimately bring forth a quantum internet, distributed quantum sensing using two-mode squeezed-vacuum states is gaining momentum, and true quantum imaging may yet emerge from quantum illumination or other paradigms. Finally, I should note that my use of “true” in conjunction with quantum imaging is there for an important reason. A quantum imager is one whose analysis necessarily requires quantum mechanics, e.g., Boston University’s quantum optical-coherence tomography (OCT), which offers a factor-of-2 axial-resolution advantage and even-order dispersion immunity in comparison with classical OCT. Then there are quantum-mimetic imagers, e.g., my group’s phase-conjugate OCT, which mimics quantum OCT using classical-state light beams with phase-sensitive cross-correlation to achieve the same benefits but with the advantage of high-brightness operation. Finally there are quantum-inspired imagers, e.g., the University of Waterloo’s chirp, anti-chirp configuration, which realizes the preceding OCT advantages in a totally different configuration. So, be willing to jump back and forth across the classical-quantum divide, not just in imaging, but also in sensing and communication. It has served me very well over the years, and I believe it will do so for you too.
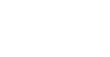

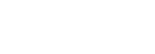

Copyright © 2022-2023 MIT Center for Quantum Engineering – all rights reserved – Accessibility